After a frustrating set of July launch delays, a decision has been made by the Dawn Project to launch Dawn in its September / October launch window.
Update: The September 27 launch was a success!
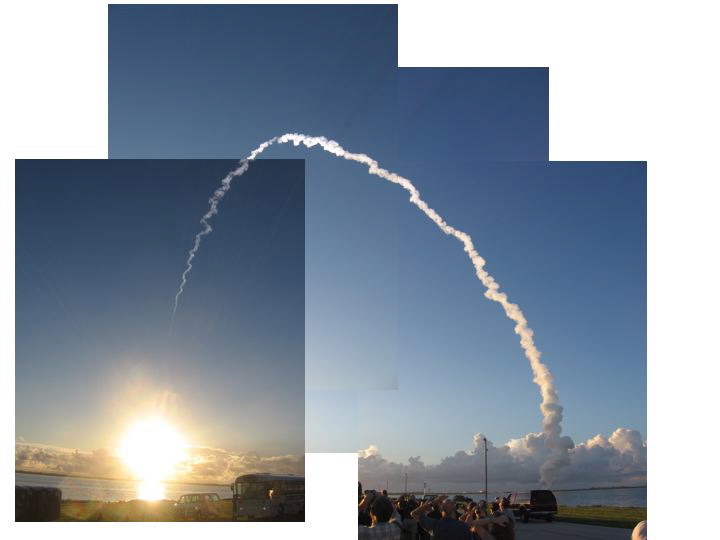
(The mosaic shows the exhaust trail from Dawn's Delta II launch vehicle superimposed on the sunrise over Cape Canaveral. Photo Credit: Randy Pollock)
What, you haven't heard of Dawn?
You are not alone. NASA's Dawn mission to orbit the asteroids Vesta and Ceres has existed mostly out of the public eye, supported by a small, but dedicated group of scientists and engineers, of whom many live and work outside of the United States, because two of the three Dawn instruments were built by NASA's foreign partners. 'Out of the public eye' for Dawn also, at times, meant literally out, because Dawn was twice canceled by NASA.
Such a rocky road of mission development seems appropriate for Dawn, however, since its goal is to investigate the early dawn of solar system evolution, out of which the asteroids and their larger cousins, our rocky (terrestrial) planets formed.
Targets
The mission asteroid/dwarf planet targets of 4 Vesta and 1 Ceres anchor the two ends of the spectrum of possibilities of the formation of planetesimals. At one end of the spectrum is 516-km diameter Vesta, a water-poor, dense (average density 3.4 g/cm3 (1)) asteroid, that has experienced significant heating to melt its interior and separate (differentiate) into a basaltic crust, a silicate mantle and an iron core, as in the terrestrial planets (2).
At the other end of the spectrum is 975-km diameter (3) Ceres, a water-rich, porous (average density 2.1 g/cm3 (1)) unmelted (?) 'dwarf planet', which, at ~9.5 x1023 g (4,5), contains about 1/3 of the total mass in the asteroid belt (~0.0005 Earth masses (6)) and is about 3.5 times more massive than Vesta, the next heaviest asteroid. Since only one-half AU separates the two bodies in the asteroid belt, one must ask: how could the two bodies be so different? To answer that question, let's journey back in time, 4.6 billion years.
Solar Nebula Temperature Profile
A basic result of the temperature profile in the low pressure solar nebula is a condensation sequence for gases of molecular substances to condense directly to form solids. Condensation of a particular species occurs when the partial pressure of that species exceeds the saturation vapor pressure. Since the latter is steeply dependent on temperature, this criterion translates into a distance from the nebula disk center at which the species is saturated. Within a few AU of the Sun, the solar nebula was too hot to vaporize all but the most refractory (i.e. elements that removed from the vapor phase at high temperatures) materials. However, further from the Sun, icy grains could form. The link between species and heliocentric distance leaves its fingerprint onto the spatial distribution of elemental abundances in the solar system. However, dynamical solar system processes and time leave higher order fingerprints of their distribution, giving us a rich dataset to extract the information of our origins.
Where is the Snow Line?
Water is one of the questions that the Dawn mission hopes to address. HST observations of Ceres in 2005 (3) suggest that frozen water might account for as much as 25% of its interior, which would be greater than all of the fresh water on Earth. This large and wet body, which appears to be covered by carbonates and clays (7), is only radially separated by 0.5 AU from smaller and dry, basaltic-covered Vesta. If we understand how Ceres could have acquired its water, then we would better understand how our own Earth acquired its water. The water condensation front (sometimes called the snow line or frost line) is dependent on the ambient temperature and pressure of the solar nebula disk and the cosmic abundance of the elements and their distribution (8). The radius of the snow line is not clearly defined, however, because it is dependent on the model of the solar nebula. In older, dusty, cold disk models, the snow line could lie at 1 AU (9), but more typical solar nebula models give about 5 AU at a given time in the disk, which then moves inward to 3 or 4 AU, as the disk ages and cools. A typical nebular temperature for the water condensation is ~170K.
The Bottom-Up Assembly Theater
The subsequent scenes in the solar nebula theater are generally assumed for the rocky (terrestrial) planets, to follow a bottom-up planet formation process, punctuated with periods of high energy events. The bottom-up assembly process (by nongravitational 'sticking' of the particles or else by collective self-gravity) from sizes centimeter to kilometer is under debate, but it must have occurred quickly because meter-sized particles would have been removed due to differences between the object traveling with a keplerian speed and the gas rotation speed in the protoplanetary disk. High energy burst events, for example, a lightning discharge, an accretion shock, or a magnetic nebular flare were necessary because the glassy chondrules embedded in chondritic meteorites and interplanetary dust particles could not have been formed in the ambient temperature of the innermost solar nebula and the cooling time was on the order of hour(s) (10).
Once kilometer-sized bodies, the planetesimals, formed, then the gas drag that was previously pulling the particles towards the Sun, was no longer a dominant effect, and gravitational forces took over. The dynamics of planetesimals moved as an elaborate dance: now the nebula brimmed with planetesimals encountering on crossing orbits similar planetesimals every ~1000 years, impacting, some shattering, some aggregating. In another 20,000 years, roughly 10,000 bodies the size of the Moon, called embryos, were formed, all orbiting in nearly circular orbits. The time for the aggregation to larger planet-sized bodies, however, is thought to be much longer than the formation of Jupiter and the dissipation of the solar nebula (11). On the way to Mars-sized embryos, the planetesimal growth became a runaway process, in which the largest body in a localized region of the planetesimal swarm gained mass more rapidly than the next largest. The mass dominated that region. Ceres might be one incipient runaway body that did not grow to completion, stopped cold. Why? In one word: Jupiter. In particular, the disturbing forces of Jupiter's gravity. Such a scenario, however, requires a precise timing for Jupiter's formation to interrupt the runaway (12).
The Jovian Giant
Jupiter has been the dominant influence on the asteroid belt over the history of the solar system, with its formation in space and time determining the clearing of the asteroid belt and possibly truncating the growth of Ceres. Scientists usually assume that a smooth distribution of protoplanet masses existed until Jupiter entered the scene, which then disrupted the aggregation process. Moon-sized bodies were ejected, some achieved Earth-crossing orbits, and eventually depleted by a factor of ~4000 the mass of the asteroid belt into its value today.
Unfortunately, the time and place of Jupiter's formation is not well constrained. Evolutionary models of Jupiter's interior and compositional data are gradually favoring a nucleated instability model for Jupiter's formation (13), which assumes that a planetary embryo formed as a result of a runaway growth out of a swarm of planetesimals in the giant planet region of of the solar nebula, then grew by accretion of large quantities of gas onto the resulting core in a time less than ~10 Myr. The locations of the original formation of the giant planets such as Jupiter, which so strongly sculpted the asteroid belt, are also under investigation. Tipped off by the observations of extrasolar planets, which seem to indicate that the extrasolar giants formed far from their star and migrated inwards (due their interaction with their gaseous nebular disk), investigators explain that such an interaction in our own solar system could have moved Jupiter slightly inwards, Saturn slightly outwards, and Uranus and Neptune significantly outwards from their formation locations. Now, what can we say about Vesta's formation?
Vesta in your hand
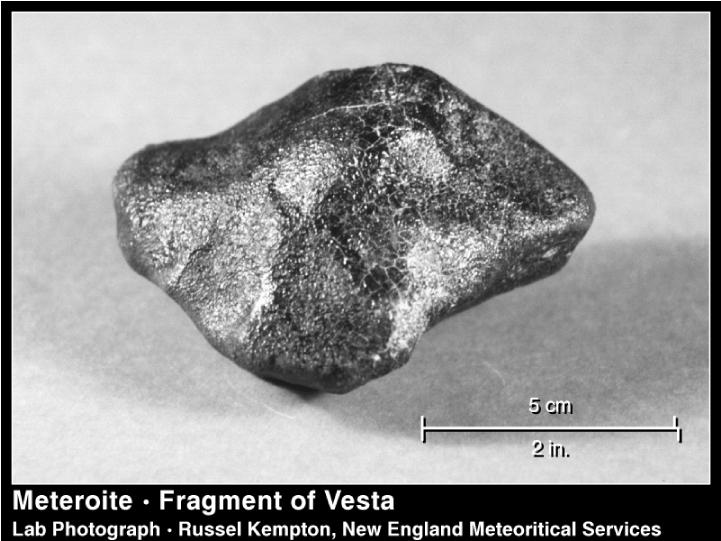
Almost forty years ago, T. McCord determined through visible and near-IR spectroscopy (2, 14), that the surface of Vesta is composed of a basaltic crust. In these last decades since, astronomers have learned that remote sensing is not the only way to study Vesta. Five percent of the found meteoritic samples on Earth, the Howardite Eucrite Diogenite ("HED") meteorites, are thought to be the result of a collision or collisions with Vesta, ejecting pieces of the crust. Most (but not all) V-type near-Earth asteroids, and some outer main-belt asteroids have spectra similar to Vesta and are known as 'vestoids', pieces of which you can find in the meteorite collections of your local natural science history museum. Our meteorite collections of Vesta's crust, then, give one ground truth to Vesta's evolution. Subsequent studies derived (2, 15) the thickness of the Vesta's crust to be at least 8 km thick.
With the exception of the large impact crater ~460 km in diameter and 13 km deep in the south pole of Vesta (2, 16), giving us our HED meteorites, Vesta's crust appears to be mostly intact. So here we have a miniature planet, which, unlike Ceres, was formed to completion: heated, melted, fractionated, and solidified. In such an active collisional environment, as what existed in the early solar system formation, a mostly preserved asteroid crust then provides a notable marker in the chronology of the evolution of the solar system. The marker tells us when the mass of accreted bodies in the asteroid region was removed, in order to produce the final asteroid belt. The mass must have been removed relatively early (12). How? Perhaps the answer is the same: Jupiter.
Some Chronometers of Solar System Formation
Vesta's crust is one of a collection of notable events with which we can anchor the time evolution of the solar system. The elaborate solar system dance of colliding bodies proceeded with its own rules of timing and location, with particular events leaving particular markers of the solar system chronology. The most valuable markers are the centimeter-sized calcium-aluminum-inclusions (an image of the CAIs), which, for 35 years, have long been considered to represent the first solid condensates, a "Time Zero", T0, for the beginning the solar system. The absolute ages of CAIs, using Pb-Pb radioactive dating are 4554 +/- 4 million years (17). The flash event that produced chondrules, which were buried in the parent bodies of the meteorites, giving the meteorites the name: chondrites, left a more diffuse marker because the smaller millimeter-sized chondrules leads to lower U/Pb ratios in the dating procedure, which are more difficult to measure. Their ages, however, are a few million years younger (18).
Upon application of the radioactive chronometers of 53Mn and 182Hf to HED meteorites, geochemists learned that Vesta probably formed and differentiated within 5 Myr after T0 (19, 20). Ceres is not as well constrained. It's formation time depends on its formation heliocentric distance, which is approximately 50 Kyr, 500 Kyr, and 5 Myr, after T0, at distances of 1 AU, 2 AU, and 2.5 AU, respectively (18). The location at which it formed probably depended on ... yes, Jupiter and Saturn (6).
What Heated the Asteroids?
We know that Vesta warmed up to melting temperatures, its interior differentiated into a layered structure, and then cooled and solidified fast. The fast cooling process is not surprising for a small, rocky asteroid in the inner solar system. Because of Vesta's small size, which gives it a surface-to-volume ratio 25 times greater than Earth's, Vesta's heat loss proceeded quickly. What is surprising for Vesta is its melting temperature. It was much hotter than it should have been, if its heat was generated by the long-lived radioactive isotopes (radioisotopes) that existed in our solar nebula (21).
Aluminum is the third most abundant element in the Earth's crust, but the twelfth most abundant element in the universe (22). Its extinct radioisotope 26Al is created in the nucleosynthesis of explosive events such as novae or supernovae, and decays to 26Mg with a half-life of only 730,000 years. Such a short lifetime is nevertheless long enough for the aluminum isotope to become embedded into the dust grains which eventually became part of the solid bodies in our solar system. Some researchers have postulated that the shock wave from the supernova that triggered the collapse of the molecular cloud out of which the Sun and planets formed, was the same event that spread 26Al uniformly throughout the solar nebula (21). Geochemists have hypothesized that the source of asteroid heating in the solar system was this radioisotope of aluminum.
Because 26Al decays so rapidly, its heating effect is large; small amounts of the isotope can greatly raise the temperature of a small body. But it's not enough to melt Vesta's interior. Given the primordial concentrations of the isotopes and the expected rate of heat loss, the radioactive isotope decay could not have melted Vesta or any other asteroid. It needed an additional mechanism. Presently the only viable alternative mechanism to add to the heat produced by radioactive decay is collisional heating, that is, the heat produced when one body collides with another. This heating mechanism is especially efficient in a highly porous body, such as the rubble pile structure of many asteroids. But is it sufficient for Vesta? The only way to know is to go to Vesta and peer inside of the craters, in order to see the surface's degree of heating and compression.
My Dawn Rocky Road Science List
Given that it is summer, and I'm located in Italy, where gelato is a larger deity than Jupiter, here is my own rocky road list of science questions that I would like the Dawn mission to help answer.- Where is the snow line and hence the emergence of water in the formation of the inner solar system?
- How common are low temperature, aqueous processes in the early solar system?
- What heated the asteroids?
- When did the heating, melting, and differentiation occur in Vesta?
- Where and when did most of the asteroids in the asteroid belt go?
- Was the growth of Ceres truncated by Jupiter? When?
- How fast is the step from asteroid-sized planetesimals to full-sized planets?
Good luck to us all for the upcoming launch and the Dawn 'odyssey'!
References
(1) McCord, T. "The Dawn Mission and Ceres and Vesta Science", presentation 6-7 February 2003, JPL.
(2) Keil, Klaus (2002), "Geological History of Asteroid 4 Vesta: The "Smallest Terrestrial Planet"" Asteroids III, W. F. Bottke, P. Paolicchi, R. P. Binzel, A. Cellino eds., University of Arizona Press, pg. 573.
(3) Thomas, P.C. Parker, J. Wm., McFadden, L.A., Russell, C.T., Stern, S.A., Sykes, M.V., Young, E.F. (2005), "Differentiation of the asteroid Ceres as revealed by its shape", Nature, Vol. 437, pg. 224-226.
(4) Pitjeva, E. V. (2005). "High-Precision Ephemerides of Planets — EPM and Determination of Some Astronomical Constants" (PDF). Solar System Research 39 (3): 176. DOI:10.1007/s11208-005-0033-2.
(5) Britt, D. T.; Yeomans, D.; Housen, K.; Consolmagno, G. (2002),"Asteroid density, porosity, and structure", in _Asteroids III, W. F. Bottke Jr., A. Cellino, P. Paolicchi, and R. P. Binzel (eds), University of Arizona Press, pg. 488.
(6) Chambers, J. (2006), "Meteoritic Diversity and Planetesimal Formation", _Meteorites and the Early Solar System II, D.S. Lauretta and H.Y. McSween Jr. eds., University of Arizona Press, pg. 494-495.
(7) Rivkin, A.S., Volquardsen, E.L., Clark, B.E. (2006), "The surface composition of Ceres: Discovery of carbonates and iron-rich clays," Icarus 185, pg. 563-567.
(8) Lunine, J. (2006), "Origin of Water Ice in the Solar System", _Meteorites and the Early Solar System II, D.S. Lauretta and H.Y. McSween Jr. eds., University of Arizona Press, pg. 309-312.
(9) Sasselov, D.D. and Lecar, M. (2000), "On the Snow Line in Dusty Protoplanetary Disks," Astrophysical Journal 528, 995-998.
(10) Ciesla, F. J. (2005). Chondrule-forming Processes-An Overview, in Chondrites and the Protoplanetary Disk, Krot, A.N. and Scott, E.R.D. and Reipurth, B., eds. ASP Conference Series 341, Astronomical Society of the Pacific, pg. 811-920.
(11) Morbidelli, A., J. Chambers, J. I. Lunine, et al. (2000), Source regions and timescales for the delivery of water to the Earth, Meteoritics & Planetary Science, 35, 1309-1320, 2000.
(12) Weidenschilling, S. and Cuzzi, J. (2006), "Accretion Dynamics and Timescales", _Meteorites and the Early Solar System II, D.S. Lauretta and H.Y. McSween Jr. eds., University of Arizona Press, pg. 474-475.
(13) Lunine, J.I., Coradini, A., Gautier, D., Owen, T.C., Wuchterl, G. (2004) "The Origin of Jupiter", in _Jupiter: The Planets, Satellites, and Magnetosphere_, Cambridge University Press, pg. 30-31.
(14) McCord, T.B., Adams, J.B., and Johnson, T.V. (1970), "Asteroid Vesta: Spectral reflectivity and compositional implications," Science, 168, pg. 1445-1447.
(15) Binzel, R.P., and Xu, S. (1993), "Chips off of asteroid 4 Vesta: Evidence for the parent body of basaltic achondrite meteorites," Science 260, pg. 186-191.
(16) Thomas, P.C. Binzel, R.P., Gaffey, M.J., Storrs, A.D., Wells, E.N., and Zellner, B.H. (1997), "Impact excavation on asteroid 4 Vesta: Hubble Space Telescope results," Science 277, pg. 1492-1495.
(17) Russell, S.S., Hartmann, L., Cuzzi, J., Krot, A.N., Gounell, M., Weidenschilling, S. (2006), "Timescales of the Solar Protoplanetary Disk", _Meteorites and the Early Solar System II, D.S. Lauretta and H.Y. McSween Jr. eds., University of Arizona Press, pg. 235-237.
(18) MacPherson, G.J. (2006), "Calcium-Aluminum-rich Inclusions in Chondritic Meteorites", _Meteorites and the Early Solar System II, D.S. Lauretta and H.Y. McSween Jr. eds., University of Arizona Press, pg. 239.
(19) Chambers, J.E. (2005), "Planet Formation", in _Meteorites, Comets, and Planets_, A.M. Davis, ed. Treatise on Geochemistry, Vol. 1, Elsevier, pg. 470.
(20) Nichols, R.H. Jr. (2006), "Chronological Constrains on Planetesimal Accretion", in _Meteorites and the Early Solar System II, D.S. Lauretta and H.Y. McSween Jr. eds., University of Arizona Press., pg. 466-467.
(21) Rubin. A.E. (2005), "What Heated the Asteroids?," Scientific American, May, pg. 62-69.
(22) Clayton, D.(2003), _Isotopes in the Cosmos_, Cambridge University Press, pg. 130-131.
Comments