Are you getting aroused yet? we are talking about a haDR-on here, don't be mistaken, but the matter is not less sexy than the stuff you'd get on the sports section anyway. For, you know, there is simply so much we still do not know about how quarks can create excited states of nuclear matter, that one cannot ignore any new development.
Just like protons and neutrons bind into atomic nuclei in ways that store enormous amounts of energy - something that was realized in the late thirties, and led to nasty as well as fantastic new technology developments - also quarks bind into hadrons much in the same way, and they, too, store ginormous energy in the bounds they make. One day that energy might become exploitable, but please do not say you heard that for me, or my career would be over. For now, though, it is just amazing to see the strip dance of the Xi_b states. But let me start from the beginning, for those of you who are not familiar with this stuff.
Background: CMS and the b quark
CMS is the acronym of the collaboration I work in. It stands for "Compact Muon Solenoid", although its participants have renamed it "continuous meetings system" for obscure reasons. It includes over 3000 scientists from all over the world, who use the detector they have built at the beginning of this century to analyze the proton-proton (and heavy ion) collisions that are produced by energetic beams in the Large Hadron Collider, the highest-energy proton-proton collider in operation, at the CERN laboratories near Geneva, Switzerland.
Proton-proton collisions of very high energy often produce quarks of the "third generation" - the heavier replica of the three light "up" and "down" quarks that make up the proton and the neutron. Quarks, in fact, come in three generations, as shown in the table below. The heaviest quarks are named "top" and "bottom". They are both extremely intriguing to study, for a variety of reasons connected with questions such as whether the Universe is stable, or it is capable to pull off the trick to quantum tunneling, annihilating everything that there is just when you finally decided to buy a new car; or why there is more matter than antimatter around, and why it matters.
For all we can tell, the bottom quark is a point-like matter particle, while the proton is an extended object; and yet, the former weights 4.3 times more than the latter. And that's peanuts if we take the top quark, the heaviest of the lot: it weighs about 180 protons by itself.
But let's focus on the bottom quark here. That particle can be produced in pairs with its anti-matter companion, the anti-bottom quark, in LHC collisions. When this happens, the pair may end up traveling away together, or each of the two quarks may be kicked away in different directions. In the first case there is a chance that the pair will create a so-called "quarkonium" state, a meson made by a quark and antiquark of the same kind. Otherwise, each of the lone (anti-)bottom quarks may find one or two companions to dress up as a hadron. That is because quarks cannot travel away from the interior of the proton-proton collision region alone, as they carry a quantity, colour charge, which cannot exist in free space.
Creating Xi baryons
So to neutralize their colour charge, the lone bottom quarks need an antiquark companion carrying the opposite colour, or two other quarks of different colour. The first scheme produces mesons - quark-antiquark bound states, and the second produces baryons, of which the proton and the neutron are the most notable examples, and of which the Xi baryon, the subject of this article, is a very special one.
The Xi_b baryon contains a bottom quark, a strange quark, and an up or down quark. Since the bottom quark is very heavy, the Xi_b also is. The combination with a strange quark makes this particle more complicated to produce than other bottom-rich baryons, so it is a very rare particle. But its decays are quite striking, as the bottom quark lives a long life, and when it decays it does so in very distinguishable ways. But the Xi_b is not the particle CMS discovered.
What CMS found was a signal of the doubly excited Xi_b particle, Xi_b**. That baryon can exist in excited states, ready to de-excite with the emission of the extra energy, is a thing that makes them even more similar to atomic nuclei than they already were. But the de-excitation results in the emission of strong force carriers - gluons - and not gamma rays (photons) as atomic nuclei emit. Those gluons eventually materialize into pions, through complex processes that materialize bound states of quark-antiquark pairs (that is what pions are).
Decays of the Xi_b**
To prove that you see a Xi_b** particle you need to see those two pions, and then detect the very striking sequence of decays that transmutes the Xi_b (now rid of the asterisks, as it has de-excited) into ordinary matter - matter, that is, made of quarks of the first generation, up and down. There are in reality two different main ways for this to happen.
The first way a Xi_b may decay is by emitting a J/Psi meson and a Xi baryon. The former is a quarkonium state made up by a pair of charm quarks, and the latter is made up of two strange quarks and a down quark. The Xi can then decay to a Lambda baryon and a pion, and the Lambda finally decays to a proton and a pion. As you see, a lot is going on: by tracking these cascade of decays the CMS physicists can reconstruct the mass of each of the intermediate particles, so the background that mimics the chain is reduced significantly.
One should also note that the J/Psi meson is seen by detecting its decay into a pair of opposite-charge muons. Not all J/Psi particles decay this way (in fact, only 6% of them do), but by selecting such decay modes is a great way to ensure that one is not fooled by backgrounds.
In the second decay mode, the Xi_b makes more of a show, as it produces a J/Psi, a Lambda baryon, and a kaon simultaneously. In reality, a soft photon may also be emitted, but that is a detail that does not belong here. In any case, the resulting Lambda baryon also successively decays to a proton-pion pair, and the decay is no less spectacular.
Graphs that will make things more clear for you
Below you can see a graph I drew to explain what is really going on in one of these decays. The thing to note is that the successive decays are mediated by the weak, the electromagnetic, or the strong interaction. The weak interaction takes place by the presence of a W boson; the electromagnetic one has the photon as its vector, and the strong interaction has the gluon as a mediator. The W can change the flavour of quarks it couples to, but the photon and the gluon cannot - that is why the chain from bottom to charm to strange to up quark is always produced by the intercession of a W; instead, the materialization of a up-antiup pair in the first diagram is due to a strong interaction.

In the diagram, which you should read left to right, each circle (okay, I am not Giotto - let's call them ellipsoids) represents a particle. Continuous lines represent the propagation of matter particles: quarks and muons (there's nothing else in the pic, although of course if there were electrons they would also get lines). Lines have an arrow that distinguishes particles (forward-going) from antiparticles (backward oriented). The dotted line is a W boson, the wiggly line is a photon, and the curly line is a gluon.
So the diagram reads: "A Xi_b comes in, and soon a weak interaction changes its bottom quark into a charm quark. The W boson materializes an anticharm-strange pair, and the anticharm merges with the charm to form a J/Psi meson; the latter then decays by annihilating the pair into a photon, which subsequently materializes a muon pair. Meanwhile the baryon has now become a (sds) one -it is a Sigma baryon although I did not labeled it as such in the diagram. One of the strange quarks in the Sigma soon emits a gluon; the gluon materializes an up-antiup pair, of which the latter merges with the strange quark to form a negative kaon. The baryon is now a Lambda, with constituents (uds). The Lambda baryon now has its strange quark emit a W boson, turning into an up quark; this up quark merges with an anti-down quark materialized by the W boson, forming a negative pion. The baryon now has a (uud) composition, and it is a perfectly stable proton.
Of all particles at the end of this decay chain, only the proton is stable: the pion, the kaon, and the muon will all decay by weak interactions, eventually yielding electrons (in total, 3 electrons and one positron) and as many as eight neutrinos.
One further thing to note is that quark lines track the colour (or anticolour) carried by these particles. By looking at the graphs you realize how you cannot destroy colour, as if you have three colour lines in the initial state, you will end up with three lines in the final state. That's reassuring: we are made of protons, and it is precisely that property that ensures the stability of our atoms!
Some graphs from the CMS analysis
Below I have seconded my urge to show you the nice "peaks" that the reconstructed masses of these particles make, in CMS data.
First of all, the baseline signal of Xi_b decays, which are reconstructed before an attempt is made at associating to these particles a couple more pion candidate tracks:
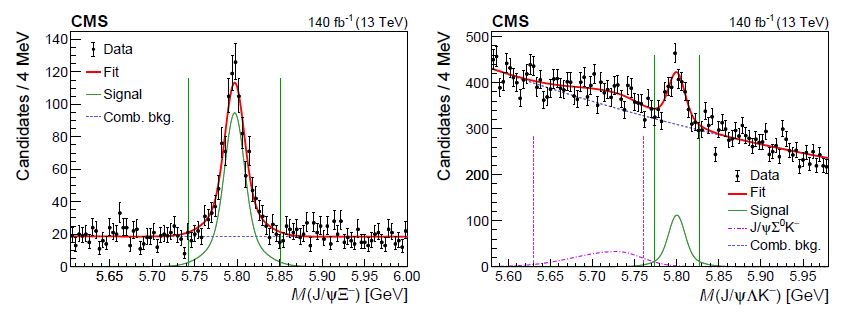
Above, the black points are CMS data, and the red curve is the fit to the sum of signal (green) and background contributions. On the left the Xi_b signal found in J/Psi Xi final states is cleaner than the one on the right, which is ridden with higher combinatorial backgrounds. In addition, a significant part of the data on the right is constituted by "partly reconstructed decays", where a photon was lost.
And then, the Xi_b**, which are extracted in the "mass difference" variable:
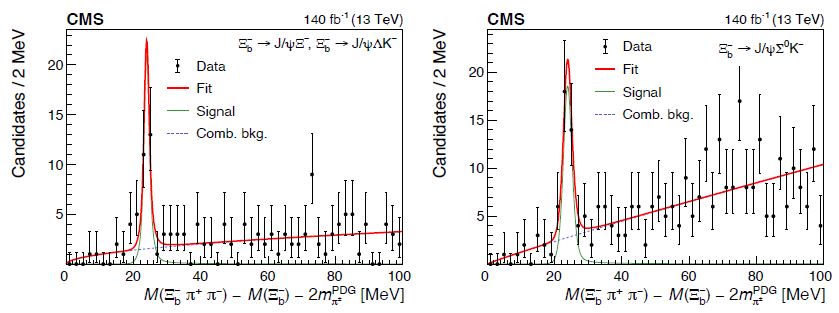
The trick of taking the combined invariant mass of all observed particles in the final state, and subtracting off the known mass of the individual bodies, is as old as Pithagoras or something like that. Here you can see that the mass difference sharply peaks at the "released energy" that is the surplus of mass-energy possessed by the decaying Xi_b particle. From these signals, CMS is capable of measuring the Xi_b** mass with sub-MeV precision.
There would be a lot to say about the signals, the backgrounds underneath, and their resolution, how they are reconstructed etcetera, but I guess that if you are curious about this information you are likely good enough for reading it directly in the preprint...
---
Tommaso Dorigo (see his personal web page here) is an experimental particle physicist who works for the INFN and the University of Padova, and collaborates with the CMS experiment at the CERN LHC. He coordinates the MODE Collaboration, a group of physicists and computer scientists from eight institutions in Europe and the US who aim to enable end-to-end optimization of detector design with differentiable programming. Dorigo is an editor of the journals Reviews in Physics and Physics Open. In 2016 Dorigo published the book "Anomaly! Collider Physics and the Quest for New Phenomena at Fermilab", an insider view of the sociology of big particle physics experiments. You can get a copy of the book on Amazon, or contact him to get a free pdf copy if you have limited financial means.

Comments