The course
My course has a duration of 64 hours, and is structured in four parts. In the first part, which usually takes about 24 hours to complete, I go over the most relevant part of 20th Century physics. We start from the old quantum theory and then we look at special relativity, the fundaments of quantum mechanics, the theory of scattering, the study of hadrons and the symmetries that lead to the quark model, to finish with the Higgs mechanism and the Standard Model.
In the second part I discuss interaction of radiation with matter, particle detection, and a few important experiments of the past, including the discovery of W and Z bosons and the charm and bottom quarks. In the third part I switch to LHC physics, describing the experiments and the search for the Higgs boson, and in the fourth and final part I give a look at searches for new physics.
Of course, the above material would be enough to fill two or three semesters worth of studies for Physics students. So how can I go within about eleven weeks through all that stuff with students that know nothing about Physics, except maybe what they learned in high school? Well, the short answer is that I try to explain the fundamental concepts, without focusing too much on details.
I do explain complex things such as isospin symmetry, Clebsch-Gordan coefficients, Goldstone bosons, or renormalization, but I make sure my students do not need to worry about having to remember everything - I am happy if they only manage to get the essential parts. This is because the course is meant to provide basic concepts that allow the student to work on data analysis from a particle collider, without feeling completely in the dark. I am perfectly aware of the fact that they -with rare exceptions- cannot possibly absorb the large amount of material of my course and make sense of it; on the other hand, I have empirically verified that my goals are usually achieved.
The experiment of teaching particle physics to statisticians was fostered by the insistence of Bruno Scarpa, a colleague in the Statistics department with whom I have been collaborating since 2014. It has in fact been working quite well, as during the past few years I had a handful of students who contributed to LHC data analysis or similar activities. We are also publishing articles with their names on it, which is a good thing for them if they want to continue with research after their thesis.
Calorimetry 101
I was saying above - and that is the main topic of this post - that I have been describing the electromagnetic calorimeter of CMS last week, among other things. I thought I would leave some trace of that discussion here, as it is a topic of relevance for Higgs physics; besides, that particular detector is one of the most remarkable calorimeters ever built, so it is intrinsically interesting to explain how it was built and why, at least broadly speaking (the details of construction choices for such a complex instrument are even above my own head, but we'll aim lower here).
So, what is a calorimeter, first of all? A calorimeter is a block of matter that is tasked with absorbing energetic particles, letting them interact with its nuclei until they lose all their energy. Heat is generated ("calor" is the Latin word for heat), but heat is very hard to measure with high precision. To give you a scale, a 100 GeV proton that deposits all its kinetic energy in a 10kg block of iron will raise the temperature of the latter by just a few trillionths of degrees (the exact number is I think 4x10^-12°, but I forgot if it is a 10kg block or 100kg... Ok it does not matter much).
Rather than heat, a calorimeter determines the energy of incident particles by counting how many secondary particles are generated in the "shower" that develops as the primary particle hits a nucleus, generating more particles that each take a share of the initial energy. Secondaries also interact with further nuclei, and the process continues with a multiplication that eventually dies out as each individual secondary particle has too little energy to create further siblings.
The general procedure to determine the energy of the originating primary particle is to detect the collective effect of all the secondaries; the number of the latter is proportional to the former. To this aim one often uses the process of scintillation: some materials yield a flash of ultraviolet light when traversed by energetic particles. More particles give more light, which can be effectively sized up by precise photomultiplier tubes.
Electromagnetic calorimeters
An electromagnetic calorimeter works exactly as I described a generic calorimeter above, but it is tasked with measuring with precision two kinds of particles (or maybe three, see below): the electron and the photon. As it moves in a material, the electron withstands strong acceleration in the vicinity of heavy nuclei, and it emits photons by the process called "bremsstrahlung". The photons thus created, if energetic enough, can also withstand an electromagnetic interaction in the vicinity of another nucleus, whereby they turn into an electron-positron pair. The latter will then repeat the procedure, so an electromagnetic shower develops.
Each photon radiation by an electron (or positron) takes place on average every time the particle has traveled by a length called "radiation length", X0, in the material. A photon will create a pair every 9/7 of a X0 instead. X0 can be a small distance (less than 6mm in lead, or a few cm in lighter materials), so we can construct compact calorimeters by using heavy elements. After a total depth of about 25 X0 -which is just 15cm of lead, e.g.- the showers produced by even the highest energy electrons and photons extinguish completely, so that there is a good proportionality between the total collected light and the incoming energy (otherwise, if some particles leaked out of the instrument carrying away energy, the proportionality would be lost).
Enter CMS
CMS stands for "compact muon solenoid" (it also stands for "continuous meeting system", but let's leave this bit for some more facetious text). In the mind of the physicists who designed it, it had to be compact because it had to do two very conflicting things:
(1) measure electrons and photons with exceptional resolution and low backgrounds, and
(2) measure charged tracks trajectories with very high resolution
If, as the original designers, you do not know the mass of the Higgs boson, you cannot go hunting for that particle if you do not get (1), as there is a mass range - when the Higgs boson is lighter than perhaps 110 GeV or so- when its decays to Z boson pairs are heavily suppressed. A signal may then best be sought for by detecting its rare decay into a pair of energetic photons. However, such a small signal is hidden in a very large background of events where two real photons are produced by background processes, or when they themselves are fake.
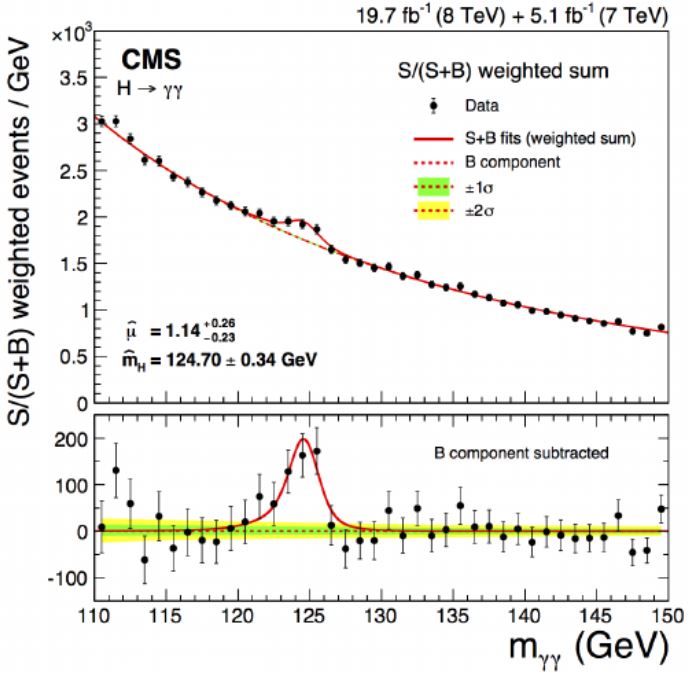
[Above, the Higgs decay to photon pairs is seen by CMS as a small bump on a large background. The narrowness of the bump depends on the high resolution on the energy measurement of photons: a twice wider peak would be much harder to put in evidence. In the top panel, the data (black points with uncertainty bars) are fit by a model (red curve) which includes an exponentially falling background and a small signal component. In the bottom panel, the signal component is shown after a subtraction of the estimated background.]
To distinguish the signal, one then must achieve the highest possible resolution on photon energy. And how do you get a high photon energy resolution? By using homogeneous calorimeters, i.e. ones where there is only active scintillator material, so that you see all the light from secondaries.
Before you measure electrons and photons in the calorimeter you must measure charged particles in a tracking system, and this has to be done within a very strong magnetic field, so that you get to curve the particle trajectories and you determine momentum from curvature - this is requirement (2). A strong magnetic field can be produced by a superconducting magnet; but if you place the magnet before the electromagnetic calorimeter, this will spoil the resolution of the calorimeter, because electrons and photons will start depositing energy in the magnet coils before they get to reach that device.
CMS designers decided to design the electromagnetic calorimeter with very dense lead tungstate, a scintillating material that could absorb showers within less than a foot of depth. This could fit inside the magnet, providing the necessary high resolution. As for the solenoid of CMS, it is the strongest one ever built for a collider detector: it is superconducting, and it produces a field of 3.8 Tesla roughly uniform over a volume of tens of cubic meters.
The above means to produce a large amount of lead tungstate to create your calorimeter. In fact, the instrument must form a 23-cm-thick cylinder around the interaction point, around the tracking volume. Its total volume is of 11 cubic meters, and the weight is of 92 tons! But the most impressive feature of this detector is its thin segmentation. The system is divided into 75,848 crystals, long parallelepipeds whose front face is only of 2.2x2.2 cm^2. This high granularity is another crucial requirement, which is aimed at reducing the background that energetic photons from Higgs decay receive... from energetic photons coming from neutral pion decay.
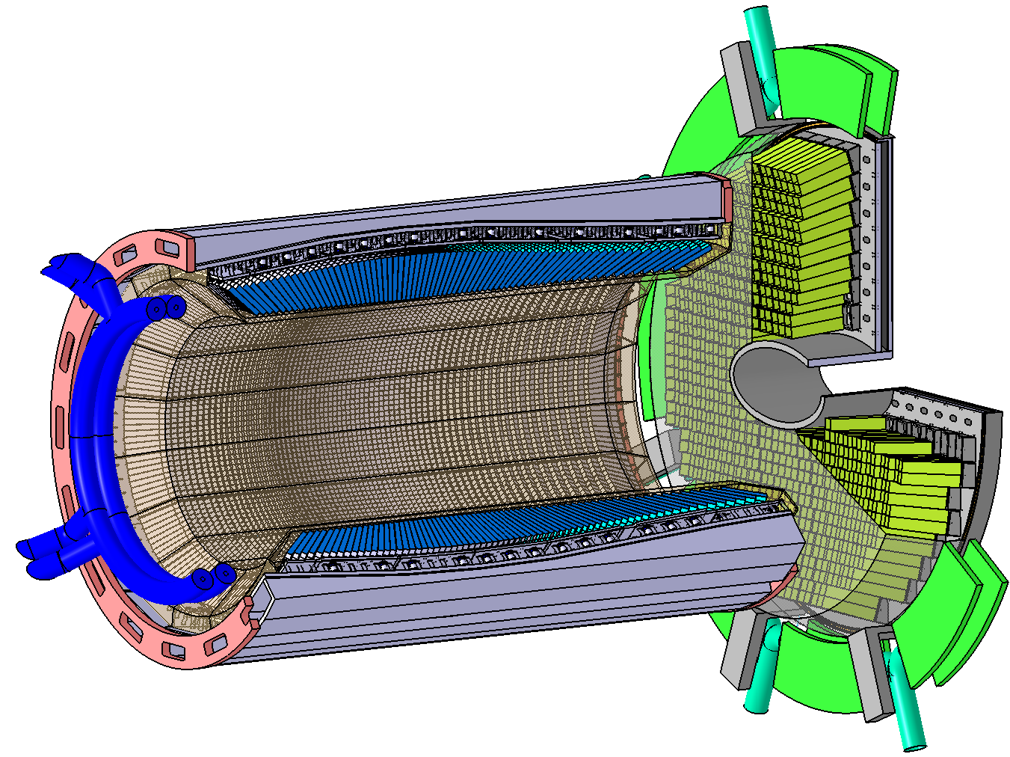
[Above, the vertex-pointing crystals of the CMS ECAL detector are shown in grey with light blue section.]
The neutral pion is the lightest quark-antiquark bound state that exists in nature. It is a member of a triplet of particles together with its positive and neutral counterparts. You get, e.g., a positive pion by binding together a up and an antidown quark, and a negative pion with a down - antiup quark. The neutral pion is a mixture of up-antiup and down-antidown. It decays by electromagnetic interaction when the two quarks annihilate. The process produces two photons.
Since the neutral pion weighs a mere 135 MeV (little more than a thousandth of the Higgs boson), the two photons it produces also carry away a thousandth of the energy, if the pion is at rest. Unfortunately, neutral pions are produced in very large amounts in proton-proton collisions, and they can be produced with arbitrarily large momentum. When that happens, the two photons will receive collectively all the pion energy, and travel away almost collinearly.
As the two photons from pizero decay hit the electromagnetic calorimeter, they may be hard to distinguish from a single well-isolated, energetic photon coming from the decay of a Higgs boson. Because of that, one needs to have as much transverse resolution as possible, such that the two independent showers produced by pizero-originated photons can be distinguished from the single shower produced by a honest-to-God Higgs decay photon.

[Above, a schematic drawing of a neutral pion producing two almost collinear photons, which hit the calorimeter at points sufficiently far from one another to give rise to independent energy deposits.]
Note that the calorimeter needs to be as far away from the interaction point as possible, in order to give the photons enough time to separate from one another - otherwise their two showers would be impossible to tell apart. This is a conflicting requirement with the one of compactness, which is called for by having a strong magnet.
Below you can see that CMS can indeed reconstruct the mass of neutral pions from the energy of independent showers produced by the two decay photons. That is what the high segmentation of the calorimeter can accomplish!
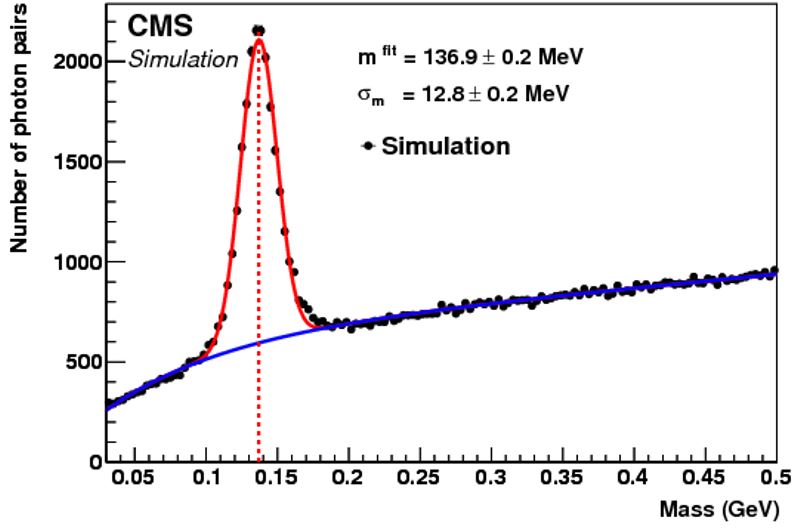
[Above, the black points indicate the rate of reconstructed photon pairs as a function of their combined mass; The red and the blue curve indicate the model of signal and background. The dashed vertical line shows that the peak sits at the nominal mass of the neutral pion, 0.135 GeV.]
So, in summary - the CMS electromagnetic calorimeter is a technological marvel, and an impressive instrument, which has been crucial in the discovery of the Higgs boson. It is however still one of the most important parts of CMS, which is now continuing the data collection in search for new physical phenomena.
---
Tommaso Dorigo (see his personal web page here) is an experimental particle physicist who works for the INFN and the University of Padova, and collaborates with the CMS experiment at the CERN LHC. He also coordinates the MODE Collaboration, a group of physicists and computer scientists from fifteen institutions in Europe and the US who aim to enable end-to-end optimization of detector design with differentiable programming. Dorigo is an editor of the journals Reviews in Physics and Physics Open. In 2016 Dorigo published the book "Anomaly! Collider Physics and the Quest for New Phenomena at Fermilab", an insider view of the sociology of big particle physics experiments. You can get a copy of the book on Amazon, or contact him to get a free pdf copy if you have limited financial means.
Comments